A Whole Energy Systems Study - The Glasgow Energy Operator
Authors
G. S. HAWKER, D. MCMILLAN - Dept. of Electronic and Electrical Engineering, University of Strathclyde, United Kingdom
G. WATSON - TNEI Services, United Kingdom
D. NEILSON - SP Energy Networks, United Kingdom

Summary
Deep decarbonisation of local energy systems requires consideration of the contributions from multiple energy resources and networks, which may not be achievable by separate Network Operators acting in isolation. In this paper, the concept of the Local Energy System Operator (LESO) is introduced, acting to bridge between different Distribution Network Operators with a Whole System view of energy provision, and able to utilise assets in both electricity and gas networks.
The LESO concept is demonstrated using a model representing the electricity and gas networks of the Glasgow City Council Area, and illustrates the ability of the LESO to optimise dispatch of assets across multiple carriers to achieve specific objectives in costs and emissions intensity, and to coordinate local investment in networks between DNOs. This bridging role is particularly appropriate to local municipalities such as Glasgow which have set ambitious near-term targets for emissions reduction, while not having direct control over the cost and emissions associated with upstream energy provision.
Scenarios representing different future upstream energy system evolutionary pathways (such as mass electrification and gas grid decarbonisation) are simulated and indicate the means by which local emissions reduction may be achieved in both operational and planning timescales via joint dispatch/planning of electricity and gas networks in order to avoid stranded assets and unnecessary sunk costs. Within operational timescales, hybrid systems such as heat networks may be dispatched to make optimal use of network headroom, which can then further inform the level of emissions reduction which will be realised through specific volumes of network investment, and where they might best be directed.
The inability of the LESO to control upstream emissions means that the rate of decarbonisation is still constrained by the emissions intensity of transmission-connected energy resources – however, as there is no implicit central planning role in deregulated energy markets, a local coordinating role is seen as key to making best use of heterogeneous regional and local energy systems, and interfacing with centralised actors to best realised the energy transition.
Keywords
Whole Energy Systems - Network Operation - Local Energy Systems1. Introduction
In recent years, the requirement to intensively decarbonise energy supply across all demand sectors (buildings, transport, industry, commerce, and agriculture) has led to the drive for a Whole Systems view of energy. An energy system may include a number of processes such as; resource extraction, refining, transportation, storage, and conversion to end-use energy services [1]. The goal of emission reduction may be best realised by joint evaluation of the contributions of different energy sources and carriers, rather than analysing each independently. In particular, views of the evolution of the electricity system are increasingly interdependent with parallel developments in the use of alternative gases within gas networks. For example, ENTSO has moved to joint scenarios for use by the electricity and gas network operators in assessing the evolution of transmission networks [2].
Within the energy system of Great Britain, electricity and gas networks are owned, operated, and regulated separately with minimal consideration given to the means by which energy service demands might be uncoupled from specific energy carriers (such as by the replacement of gas boilers with heat pumps). This is in contrast to the government-supported growth in ‘Local’ and ‘Community’ energy systems [3].
In this paper, we evaluate the means by which, joint operation of gas and electricity distribution networks may be conducted by a ‘Local Energy System Operator’ (LESO), and assess the benefits which may result. This paradigm is demonstrated for the Glasgow City Council area in Scotland, which is a Local Authority seeking to rapidly decarbonise towards Net Zero emissions. A model was produced to aid in the planning of investments by the LESO, and potentially provide a simulation of how the LESO could operate these assets to minimise carbon emissions, and maximise return on investment.
2. Background
As the proposed idea is fairly radical in nature, it follows that there is a significant demand for innovation with regards to our energy networks. Before proceeding to discuss the method, it is necessary to justify such a radial change, and provide our view the role of the LESO for discussion i.e. where this entity would potentially fit in amongst the existing energy system stakeholders.
2.1 The future of the Scottish energy system
In September 2019, the Scottish Parliament unanimously passed a Climate Change Bill [4] which increases the ambition for emissions reduction to a Net Zero target for 2045, with interim targets of 75 % in 2030 and 90 % in 2040. The Net Zero target is 5 years ahead of the same goal for the UK as a whole, recognising the increased rate at which Scotland has succeeded in reducing the carbon intensity of electricity through use of renewables (predominantly onshore wind).
One of the major ramifications of this new target is that it significantly moves forward the date by which energy use in buildings must be decarbonised. This was previously considered a marginal sector, seen as key to long-term emissions reduction but not an immediate priority, it is now likely that the residential and commercial uses of energy in buildings must be almost entirely decarbonised in order to meet the 2030 target.
The use of natural gas, predominantly burned in gas boilers for space heating and hot water provision, constitutes a large component of urban energy use and associated emissions. The ongoing use of natural gas is not compatible with the Net Zero targets. Previous studies have indicated two main trajectories, which are not mutually exclusive, for replacing the use of natural gas in heat provision [5]:
The first was the replacement of gas boilers with heat pumps, either on a per-household basis or via heat-pump powered district heating, alongside ongoing deep decarbonisation of upstream electricity supply. The second was the decarbonisation of the gas system via the replacement of natural gas with hydrogen and/or biogases.
While the transition of the gas system is likely to span multiple decades due to the need to transform supply, network, and end-use technologies (see, for example, the work proposed in the Acorn project to inject hydrogen into the Scottish gas network from 2023 [6]), the electrification option is likely to generate the near-term opportunities to meet a 2030 target and onwards towards Net Zero. However, electrification of heating places a significant additional volume of energy demand onto electricity networks which may already be highly constrained. At the peak of the ‘Beast from the East’ cold weather event in March 2018, gas use across Great Britain peaked at 214 GW, 4 times the simultaneous electricity demand of 53 GW [7].
The result of both these constraints on the electrical and gas networks points indicates a potential solution may be a ‘hybrid’ option in the supply of energy services. Whereby a single demand for energy (e.g. space heating) can be provided through different technologies i.e. it becomes possible to switch heat demand between energy carriers (such as electricity and gas) in response to local constraints/congestion, price, and emissions intensity. This concept was demonstrated in Wales through the recent Freedom project [8]. If this solution is pursued the control of when to switch between carriers should be within the remit of the LESO.
2.2. The Glasgow city energy system
Glasgow is the largest city in Scotland, with a population of around 615,000 within the City Council Area. Immediately following the Scottish Climate Change Bill, in September 2019 the City Administration Committee set a goal for Glasgow to be ‘Carbon Neutral by 2030’ [9].
2.2.1. Electricity
The City is served by 14 Grid Supply Points (GSPs) connected to the Great Britain Transmission network at 132 kV and 275 kV. The distribution network is owned and operated by SP Electricity Networks (SPEN). Specific detail on the Glasgow network is given in the methodology.
2.2.2. Gas
The majority of properties in Glasgow are connected to the low-pressure gas distribution network owned and operated by SGN, one of the Gas Distribution Network Operators in GB. Currently the network is entirely supplied with natural gas (methane), but evaluations are underway assessing the potential for hydrogen or biogas, or a blend of both, to displace methane and permit ongoing use of the gas networks under low carbon futures.
The ‘Iron Mains Replacement Programme’ is a Health and Safety Executive (HSE) led project, running since 2002, to improve the safety of ageing gas distribution network. By 2032, all iron gas distribution pipes will have been replaced with polyethylene, which are suitable for the transport of hydrogen. However, the gas transmission network is primarily composed of steel pipeline which is subject to embrittlement when exposed to pure hydrogen. Therefore, at present, the gas transmission system is only suitable for natural gas, biogas, or blends that contain a low percentage of hydrogen. A 3% hydrogen blend is expected to be achievable with little or no disruption to end users.
Demonstration projects are currently being developed which intend to operate existing gas network assets with blended gas. HyDeploy, for example, will utilise the improved gas distribution network and demonstrate a 20 % blend in the gas distribution network. It is worth noting the 20 % limit in this case was caused by limits of the end-use equipment as oppose to the gas network itself.
2.3. The role of a local energy system operator
The impacts of decarbonisation policy have led some municipalities to attempt to address the issue on a local basis. Some local authorities have established their own energy retailers, such as Bristol Energy and Nottingham’s Robin Hood Energy. However, these are only commercial entities involved in trading and retail of energy, and are not involved in network operation and planning.
In evaluating the potential role of an LESO, it is important to clarify the distinction between energy carrier demands, such as a given demand for electricity by a consumer at a point in time, and energy service demands which are the abstracted definition of the use(s) to which the energy is put by the consumer. For example, a consumer charging an electric vehicle may be considered to have different demands:
- An instantaneous demand for electricity created by activating the charger (service),
- A demand to charge the battery with a fixed number of kWh over a given time period (service),
- A requirement for the battery to have a specified minimum state of charge by a specified point in time (carrier – a fuel cell electrolyser could provide this energy),
- A desire to be able to make a specific set of journeys using their vehicle (carrier),
- A desire to be able to make a specific set of journeys by any means (carrier).
Each level of abstraction represents an increasing derogation of responsibilities and behavioural control from the consumer to the energy operator. With the difference between service and carrier now clear, the objectives of the LESO can be described as the following, in order of priority:
- To meet all demands for both heat and non-heat energy services within the greater urban area, to a high degree of probability, within the constraints of the electricity and gas networks in use,
- Where security is not compromised, to prioritise the use of lower carbon energy carriers and employ conversion technologies in meeting demand, with the remit of reducing the overall carbon footprint of the municipal area,
- To maximise the co-benefits of energy system optimisation, such economic operation to reduce fuel poverty and otherwise provide benefits to the health and well-being of consumers.
To meet these objectives, the LESO must undertake some degree of both energy system planning and operation.
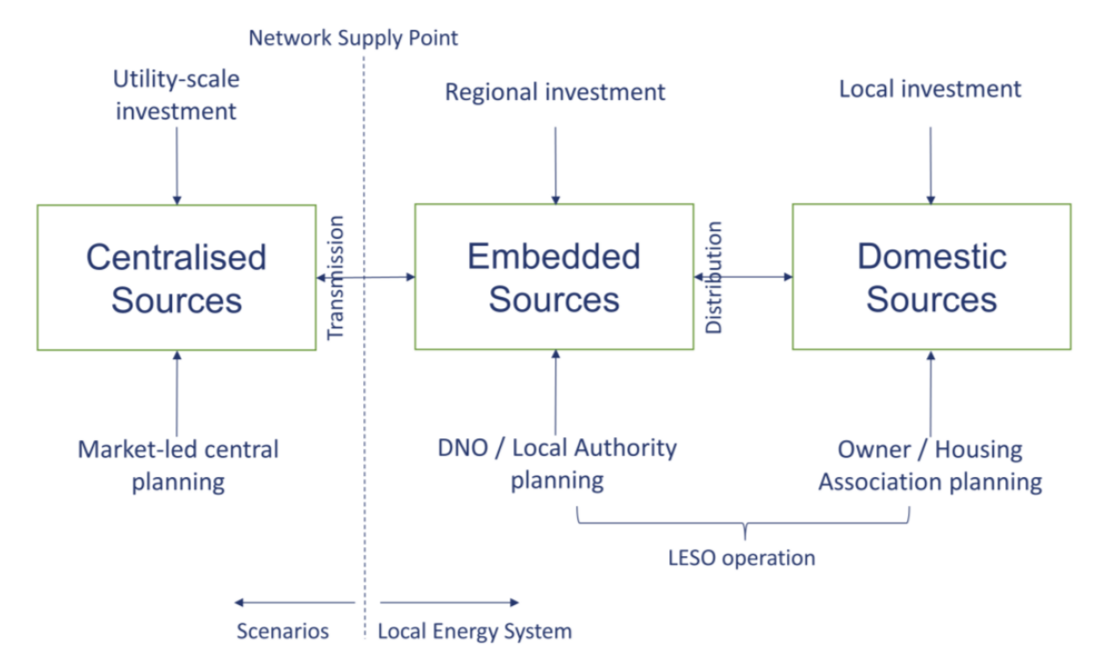
Figure 1 - Delineating the Scope of the LESO with respect to Energy Sources and Other Owners/Operators
2.3.1. Energy system planning
As shown in Figure 1, an LESO could co-ordinate with the DNOs and those responsible for planning of local housing and associated energy assets, determining the optimal way to meet current and future energy service demands given local assets and networks, within the constraints set by the emissions and costs of energy supply. The model created as part of this paper has a ‘planning mode’ to aid in making investment decisions.
The objective of the model is to undertake a higher-level approach that would consider the rating limitations of the power system, and perform studies using time-series data to reach a set of investment proposals for further consideration. The DNO would receive connection applications for any proposals brought forwards which would then undergo more detailed scrutiny from a power systems point of view (reactive dispatch, harmonic injection, dynamic and protection studies etc).
Essentially, the initial proposals consider the electricity network at a higher level, which is then refined later by the DNO as projects begin to progress through development stages. This is considered the ideal solution as the DNO best understands the details of the local power system.
2.3.2. Energy system operation
While it may seem like an LESO would not be able to perform operational duties without undertaking a role already addressed by other entities such as a Distribution System Operator (DSO), or an Electrical System Operator (ESO). It is in fact perfectly possible and reasonable for these entities to co-exist and co-operate. The role of the LESO as visualised in this paper is to be the representative of the consumer and, as such, ultimately acts as a controller of local energy demand. This could take the form of district heating systems, or a utility scale electricity to gas conversion site.
Ultimately, the levers controlled by the LESO would be local energy generation, demand, conversion, and storage. The LESO would try to optimise the use of these levers to reduce carbon footprint. It is envisaged that the LESO would own all assets that it controls, or otherwise provide financial incentives for private entities to participate. The model created as part of this paper has an ‘operational mode’ to aid in making day-to-day operational decisions.
It is worth stating that this is an experimental exercise, and there should be frank and open discussion about the boundaries between entities and future responsibilities of said entities. It is hoped that this paper will form the beginning of a conversation on this concept.
3. Methodology
3.1. A power system model of Glasgow
The power system model is based on a reduced version of the detailed local DNO power system model (the SPEN model), as shown in Figure 2. The following assumptions were applied to the power system to reduce the scope to ‘local power flows’ within the Glasgow Council area:
- All 33 kV substations must be located within the Glasgow Council district area (e.g. Node 1 is outside of district area),
- All 33 kV substations must be connected to at least one other substation within Glasgow (e.g. Node 3 substation is within Glasgow district, but connected via circuits outside Glasgow),
- The nearest (electrically) 132/275 kV substations must be considered to model local flows despite being outside the municipal area (e.g. Node 2),
- For the purposes of local energy flows, 132/275 kV substations are essentially considered to be connected without resistance (a single infinite source),
- When considering cable power rating, the lowest rated cable section i.e. the bottleneck, is considered to be the power limit of the whole line.
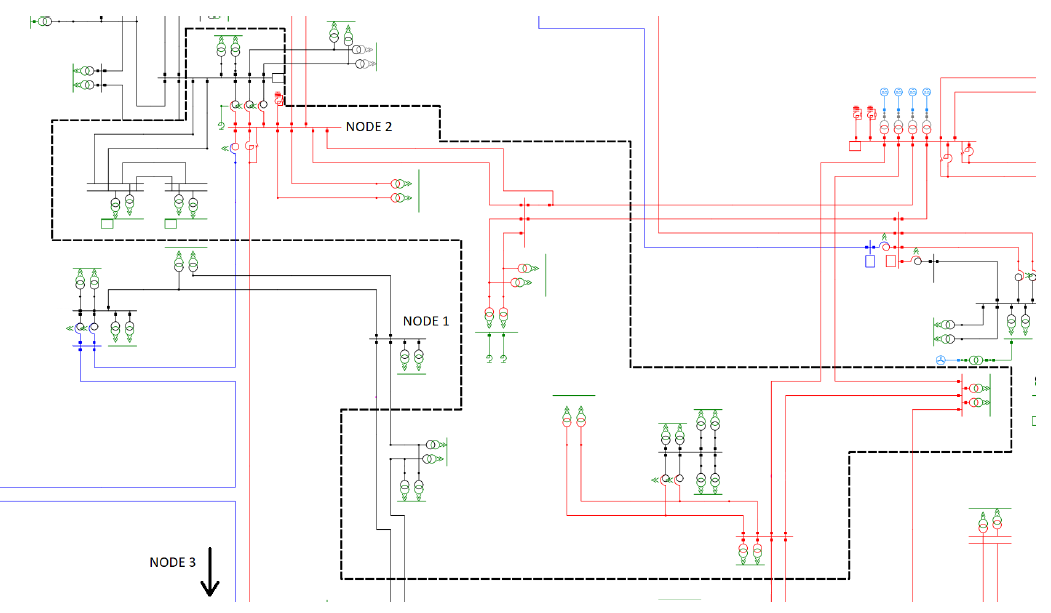
Figure 2 - Single Line Diagram of the Glasgow Council District Area Power System
In accordance with the outlined methodology, the data used from the SPEN model was as follows:
- Above 33 kV,
- The 275 kV feeder cable power rating, and 275/33 kV transformer power rating,
- The 132 kV feeder cable power rating, and 132/33 kV transformer power rating,
- Below 33 kV,
- The 33kV feeder cable power rating, and 33/11 kV transformer power rating;
- For each generator; connected GSP, rating, and power factor;
- For each load; connected GSP, rating, power factor, and load type (e.g. commercial).
This data also accounted for seasonal flows, with different power ratings extracted for summer, spring/autumn, and winter.
3.2. A whole energy system model of Glasgow
In order to investigate the different impacts of different future energy scenarios (detailed in the following section), a nodal simulation model representing the locally grouped demand and supply options was created. The model is constructed in the Calliope energy modelling framework [10] and consists of the following elements illustrated in Figure 3:
- A set of demand nodes, each corresponding to the grouped demand at each GSP.
- Time series of energy service demand for space heat and hot water for residential properties, sampled from a set of several thousand different properties, generated via a stochastic behavioural model coupled with a thermal building envelope [11], aggregated and scaled to the peak demands within the SPEN model and published gas distribution network consumption data.
- A set of indicative commercial and industrial demand profiles similarly scaled to the peak demands.
- A merit order of generation with time series generated from historically published data for transmission-connected sites in Scotland.
- MVA ratings for electricity network connections between each node taken from the reduced SPEN model.
- An unconstrained gas network with supply either represented as fixed emission natural gas, or injected hydrogen/biogas with a time-variant emissions intensity.
- Optional additional local energy assets including; electrical and thermal storage, local heat networks using either large-scale heat pumps and/or gas CHP, and embedded renewables in the form of rooftop solar PV.
Figure 3 shows the electricity supply and networks to the left, gas supply and networks to the right, with the LESO objective function at the top and energy service demands at the bottom. Dashed entities represent areas of the energy system which may fall under the remit of different entities interfacing with the LESO, such as the gas/electricity DNOs, and local energy asset operators such as CHP and storage facilities.
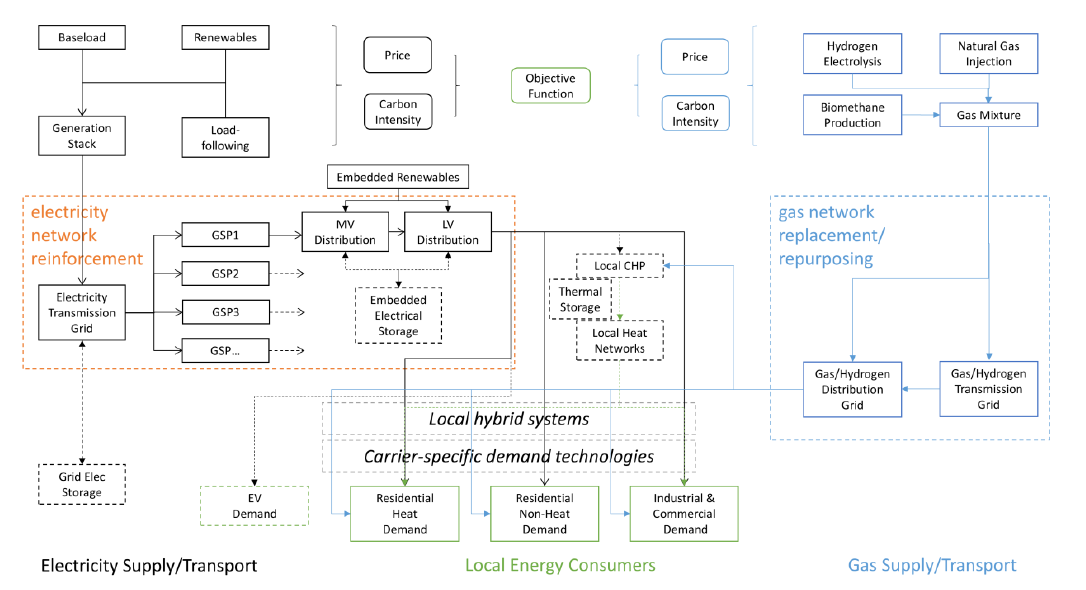
Figure 3 - Overview of the Glasgow Energy System Model
The LESO would consider the following aspects when performing the optimisation:
- A set of projected energy service demands from residential/industrial/commercial consumers over a given time horizon,
- Access to energy from electricity and gas transmission networks with time-variant prices and emissions intensities,
- Acting as a ‘price-taker’ with no impact on electricity or gas prices or intensities,
- Access to local energy resources embedded and dispatchable within those networks,
- [in planning mode] Costs of network reinforcement (defined per-kilometer for each voltage level);
The LESO would optimise considering the following constraints:
- [in operational mode] Constraints imposed on the transport of energy by the capacity of local electricity and gas networks,
- Constraints on the total emissions associated with energy supply (both upstream and local).
With the listed constraints taken into consideration, the LESO would perform the optimisation with the intention of minimising the costs associated with meeting the energy service demands.
3.3. Scenarios
An example of the way an LESO could utilise the model for the assessment of different scenarios follows.
Baseline: The current electricity network without reinforcement, alongside utilisation of natural gas defined by the current proportion of households reported as using gas heating [11].
Electrification: A 2030 scenario with a highly decarbonised electricity system, with electricity network investment to constrain total emissions to 75 %, and infilling with natural gas, based on heating fulfilled by hybrid heat pump systems. Space heating demand is reduced by additional modelling runs which incorporate building efficiency improvements.
Electrification + Storage: The network capacities generated by the previous scenario is supplemented by optimal volumes of electrical (battery) storage and thermal (hot water) storage to further utilise the time-variant emissions intensity of supply and further reduce total emissions.
Alternative Gases: A long-term scenario where the gas grid is supplied with hydrogen from Steam Methane Reformation (with CCS) supplemented by Electrolysis from renewables – the emissions intensity and cost of gas hence being variant in time, in a similar manner to the electricity network. The LESO here optimises between the costs and intensities of the two systems.
4. Results
Figure 4 illustrates the electrification scenario for a single GSP – Drumchapel – where the utilisation of heat pumps to meet space heating demand is preferable (on an emissions basis) to use of natural gas condensing boilers, but is constrained by the capacity of the primary transformer at 120MVA. Assuming the presence of a hybrid heat network to serve this area, the LESO can preferentially utilise electricity to meet heat demand where the network headroom exists, and infill remaining demand from natural gas. By electing to minimise emissions through local control of heat buffering and storage, the proportion of gas used is reduced significantly within the network constraints, while allowing further interpretation of the level of emissions reduction that would be achieved through future electrical network reinforcement.
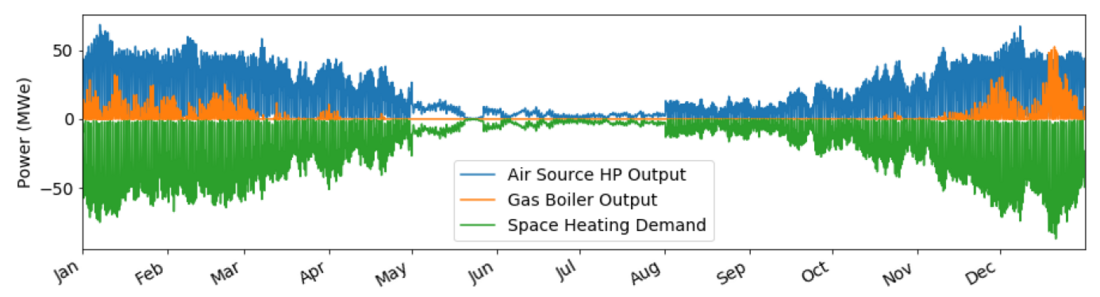
Figure 4 - Example dispatch of hybrid heating options for the Drumchapel GSP
For all the given scenarios, the simulation was run for a full year at an hourly resolution to determine the total cost of energy supply (given as £/kWhe) and the carbon emissions intensity of that energy (given as gCO2/kWhe).
The uncertainty bands given relate to different assumed costs and emissions in installed technologies, as well as for upstream energy supply. As indicated in Figure 5 each of the above pathways gives a clear route for the LESO to realise local energy service supply within emissions constraints.
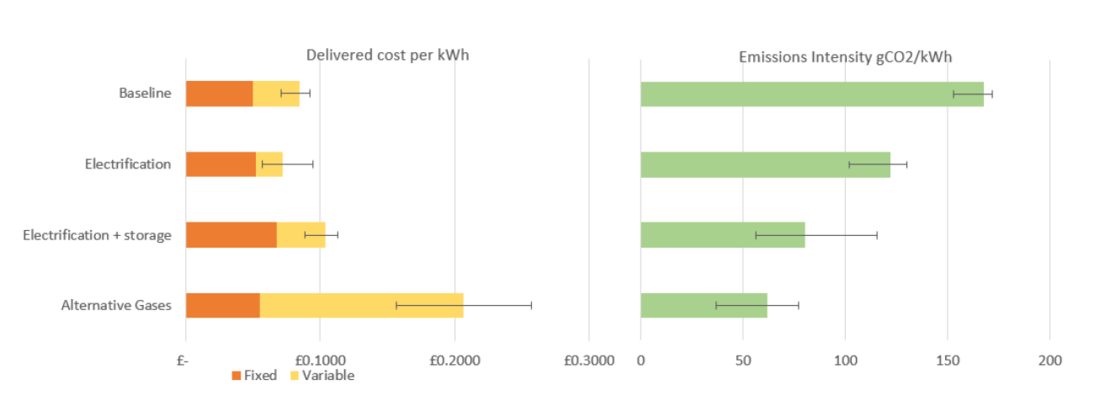
Figure 5 - Cost of Delivered Energy and Emissions Intensity Associated with Each Scenario
Under the electrification scenario, a modest investment in local networks realises and significant increase in access to low-cost renewables and an associated reduction in emissions intensity. Similarly, increasing utilisation of local storage assets increases costs but reveals further decarbonisation potential, which may be included in local decarbonisation policy. Finally, for alternative gas use, a significant emissions reduction is seen but at a large cost due to the expense of generating upstream hydrogen.
However, it should be noted that the inability of the LESO to control upstream emissions means that the rate of decarbonisation is still constrained by the emissions intensity of transmission-connected energy resources, and in this respect further coordination would be required between the LESO and the System Operators to realise deep decarbonisation.
5. Conclusions
The proposed role of a Local Energy System Operator can, by optimising the use of local energy assets across multiple energy carriers, achieve greater reduction in costs and emissions than for operators with control and view of individual carriers. This can be achieved through both operational timescales, by use of hybrid options within the local system, or on planning timescales by optimising the evolution of different networks in parallel rather than in isolation, avoiding stranded assets and unnecessary sunk costs.
This is of particular importance in the case of energy systems where hybrid technologies – switching between local use of electricity and gas –may be required to enable the transition to Net Zero emissions targets in order to prevent the utilisation of low-carbon energy sources lagging behind the capacity of the networks required to transport the energy. The LESO is well-placed to enact local decarbonisation policy and to ensure that the correct balance between cost of network and energy supply is balanced with the emissions associated with energy services, and that a successful transition is achieved over the coming decades.
The scenarios above indicate the need for a coordinating role in achieving best use of different parallel network assets, within the context of upstream energy source evolutionary pathways and uncertainty. While this could be achieved via an entirely centralised system planner, the local heterogeneity of demand and technology opportunities show that this role might best be enacted regionally and locally, making best use of available nearby systems.
References
- Pfenninger, S., Hawkes, A., & Keirstead, J. (2014). Energy systems modeling for twenty-first century energy challenges. Renewable and Sustainable Energy Reviews, 33, 74–86. https://doi.org/10.1016/j.rser.2014.02.003
- ENTSO-E & ENTSO-G, TYNDP 2020 scenarios report, https://www.entsos-tyndp2020-scenarios.eu/
- Climate XChange, 2013. Community Energy in Scotland: the Social Factors for Success https://www.climatexchange.org.uk/research/projects/community-energy-in-scotland-the-social-factors-for-success/
- Scottish Parliament, Climate Change (Emissions Reduction Targets) (Scotland) Act 2019 https://www.parliament.scot/parliamentarybusiness/Bills/108483.aspx
- UK Energy Research Centre (UKERC), 2018. Heat decarbonisation challenges: local gas vs electricity supply http://www.ukerc.ac.uk/publications/local-gas-demand-vs-electricity-supply.html
- Pale Blu Dot, ACT Acorn Feasibility Study, March 2018. http://actacorn.eu/sites/default/files/ACT%20Acorn%20Expansion%20Options%20Report%201.0%20Rev_0.pdf
- UK Energy Research Centre, Challenges for the Decarbonisation of Heat: local gas demand vs electricity supply winter 2017/18, August 2018. http://www.ukerc.ac.uk/publications/local-gas-demand-vs-electricity-supply.html
- Freedom Project, Final Report, October 2018 https://www.wwutilities.co.uk/media/2829/freedom-project-final-report-october-2018.pdf
- Glasgow City Council, The report and recommendations of the Glasgow City Council’s Climate Emergency Working Group, September 2019 http://www.glasgow.gov.uk/councillorsandcommittees/viewDoc.asp?c=P62AFQDN0GZ30GT1NT
- Stefan Pfenninger and Bryn Pickering (2018). Calliope: a multi-scale energy systems modelling framework. Journal of Open Source Software. doi: 10.21105/joss.00825
- Graeme Flett and Nicholas Kelly (2017). A disaggregated, probabilistic, high resolution method for assessment of domestic occupancy and electrical demand. Energy and Buildings, 140, pp171-187. https://www.sciencedirect.com/science/article/abs/pii/S0378778817302591?via%3Dihub
